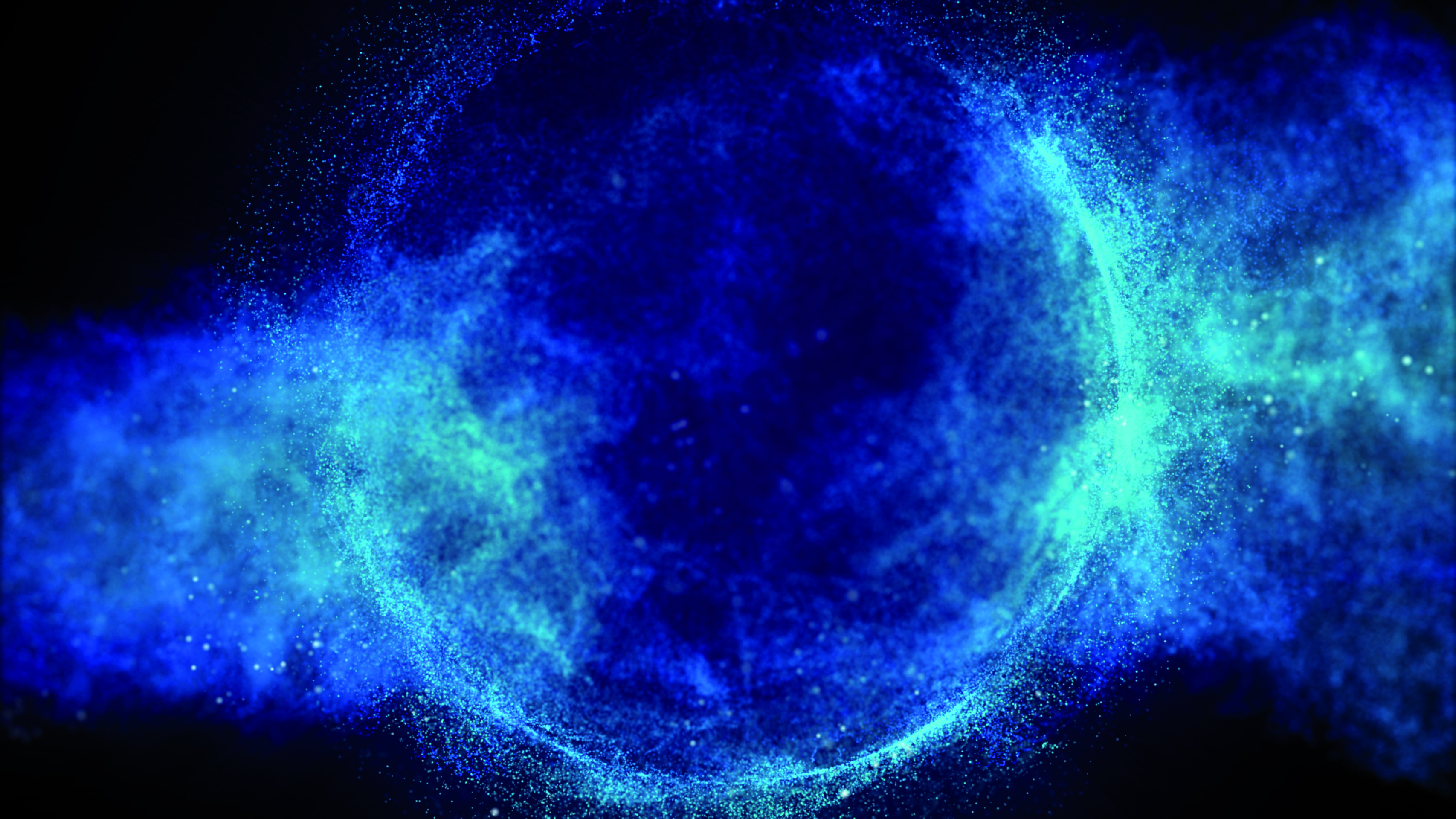
In 10 years of physics at the LHC, the particle physics landscape has greatly evolved. Today, an integrated Future Circular Collider programme consisting of a luminosity-frontier highest-energy lepton collider (FCC-ee) followed by an energy-frontier hadron collider (FCC-hh) promises the most far-reaching particle physics programme that foreseeable technology can deliver.
A complete overview of the FCC physics potential is presented in the FCC Conceptual Design Report (FCC CDR) volumes 1–3 that can be downloaded HERE.
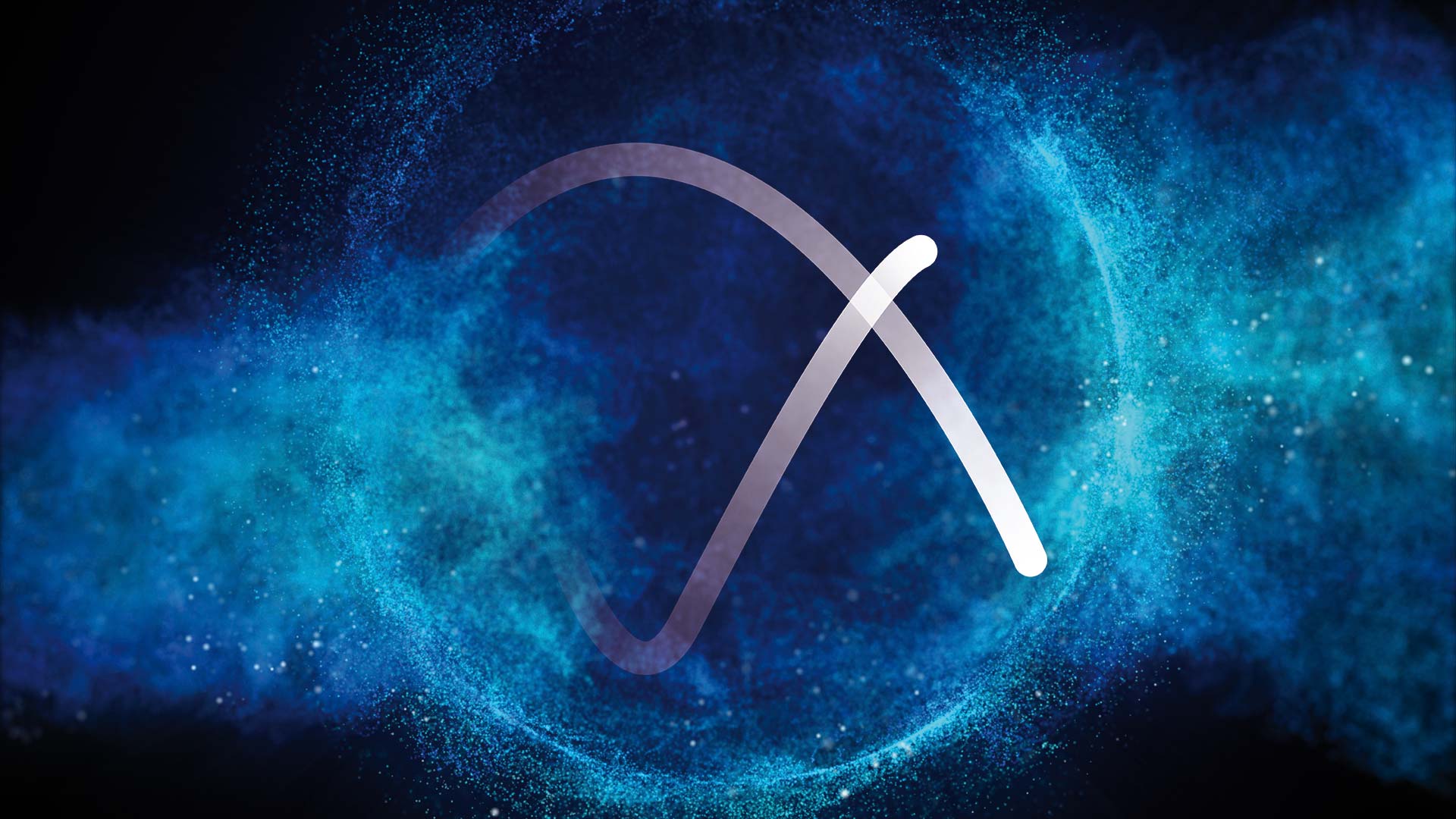
What are the fundamental interactions that govern nature?
Almost everything in the universe can be explained in terms of four fundamental interactions. They can explain the shape and structure of a galaxy due to the precise motion of the various particles governed by the laws of gravity or the structure of the molecule of water based on the principles of quantum mechanics that in turn dictate the laws of chemical bonding.
Our best understanding of how these particles and three of the fundamental forces are related to each other is encapsulated in the Standard Model of particle physics.
Fundamental interactions are characterised on the basis of the following four criteria: the types of particles that experience the force, the relative strength of the force, the range over which the force is effective, and the nature of the particles that mediate the force.
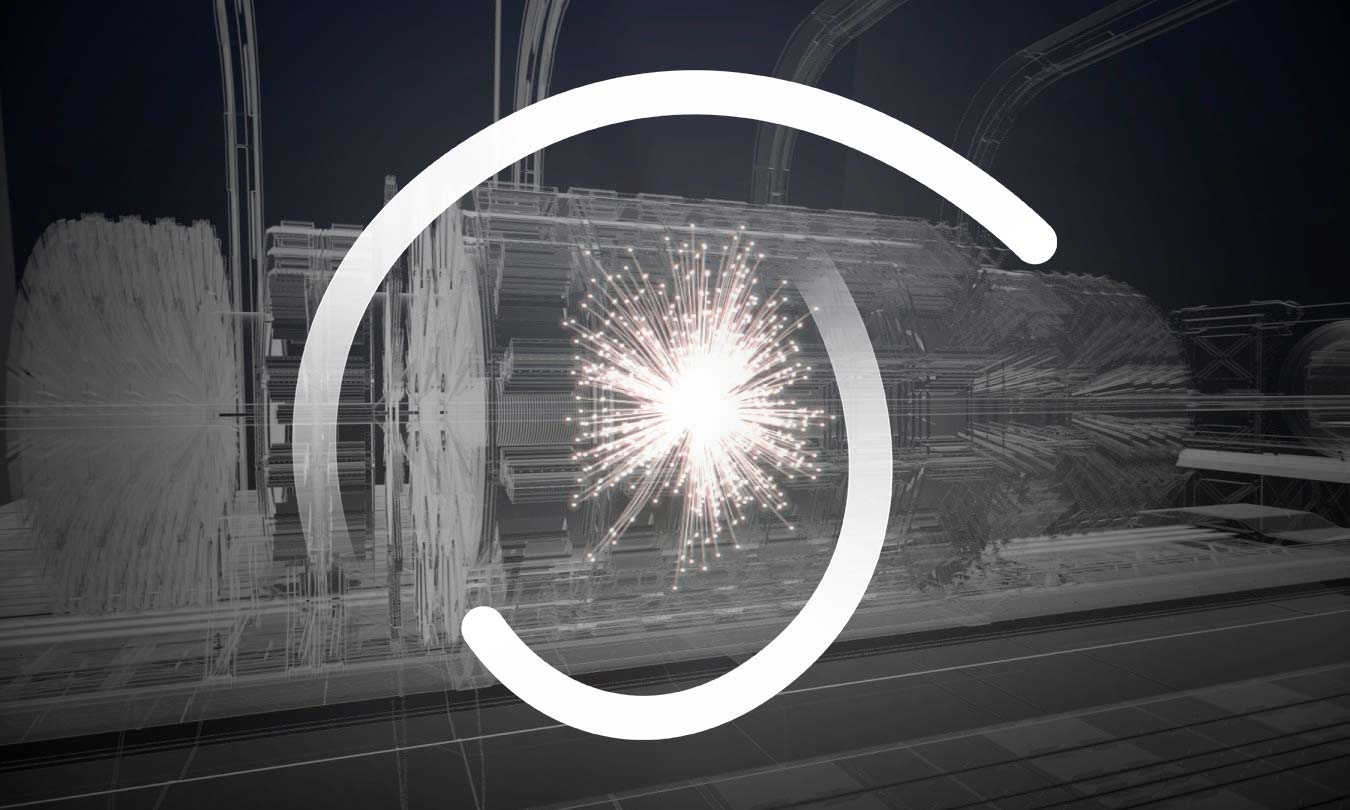
The Standard Model and beyond...
The Standard Model (SM) of Particle Physics is currently our best theory to describe the basic building blocks of the universe which are elementary particles and how they interact. It describes how particles called quarks (which make up protons and neutrons) and leptons (which include electrons) make up all known matter. It also explains how force carrying particles, which belong to a broader group of bosons, influence the quarks and leptons. Its last building block, the Higgs boson, was discovered at CERN in 2012.
The Higgs boson explains how all other particles get their masses. It was discovered by the ATLAS and CMS experiments, almost fifty years after it was theoretically proposed. Understanding its fundamental properties is one of the experimental goals for future colliders.
The Higgs’ connections to many of the deepest current mysteries in physics mean the Higgs will remain a focus of activities for experimentalists and theorists for the foreseeable future.
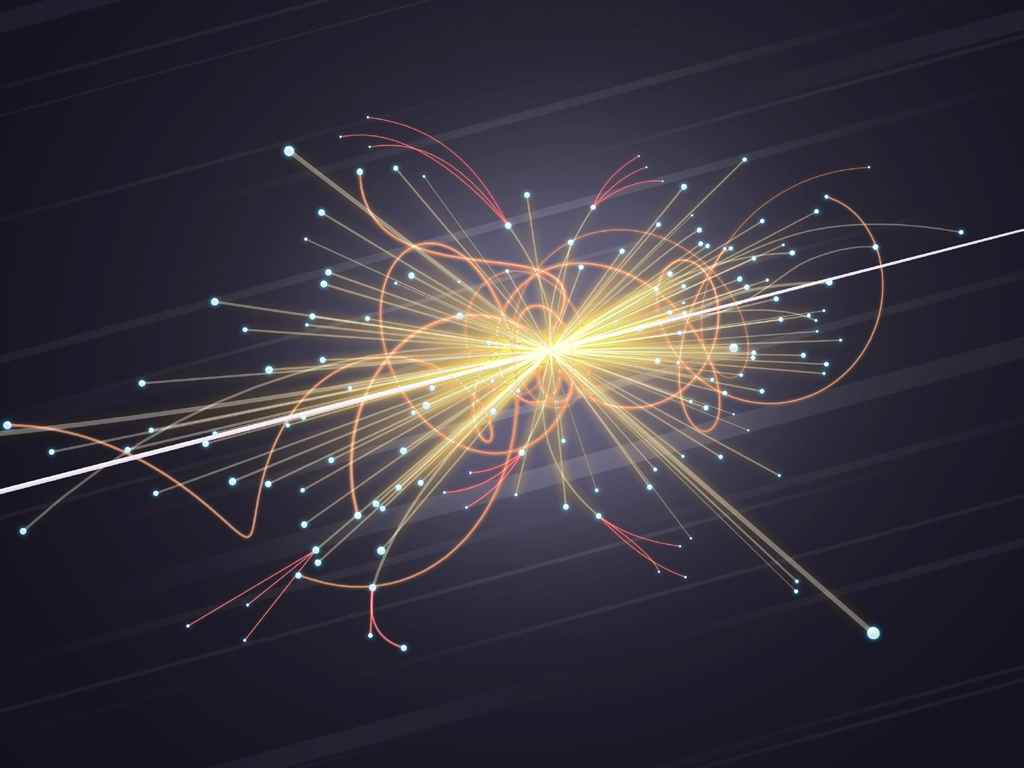
Scrutinizing the Higgs boson
The LHC has been very successful for initial exploration of the interactions of the Higgs boson with quarks, leptons and the force carries - namely W and Z bosons. One of the main goals of the FCC programme is to promote these measurements to the next level, up to an order of magnitude further in precision. Measurements of the production and decay, of the copious Higgs bosons and other SM particles produced at FCCs, could probe potential deviations from the SM predictions. They would also shed light on the fundamental nature of the Higgs boson, answering whether it is an elementary or a composite particle.
The Standard Model also predicts the self-interaction of the Higgs boson. Measuring how the Higgs interacts with itself requires much higher statistics beyond what is accessible at the LHC. FCCs could conclusively establish the existence and properties of the Higgs self-interaction, a crucial milestone given how much of nature is underpinned by it. The determination of the Higgs potential at FCC, has implications for a multitude of fundamental phenomena, such as the nature of the electroweak phase transition in the early universe.
With the Higgs boson positioned at the cross-roads of such diverse interactions, its study also offers a remarkably promising avenue for uncovering clues as to what may lie beyond the Higgs. The FCC integrated programme, with its breadth of measurements, its precision, and the huge number of Higgs produced, provides the best possible tool for this exploration.
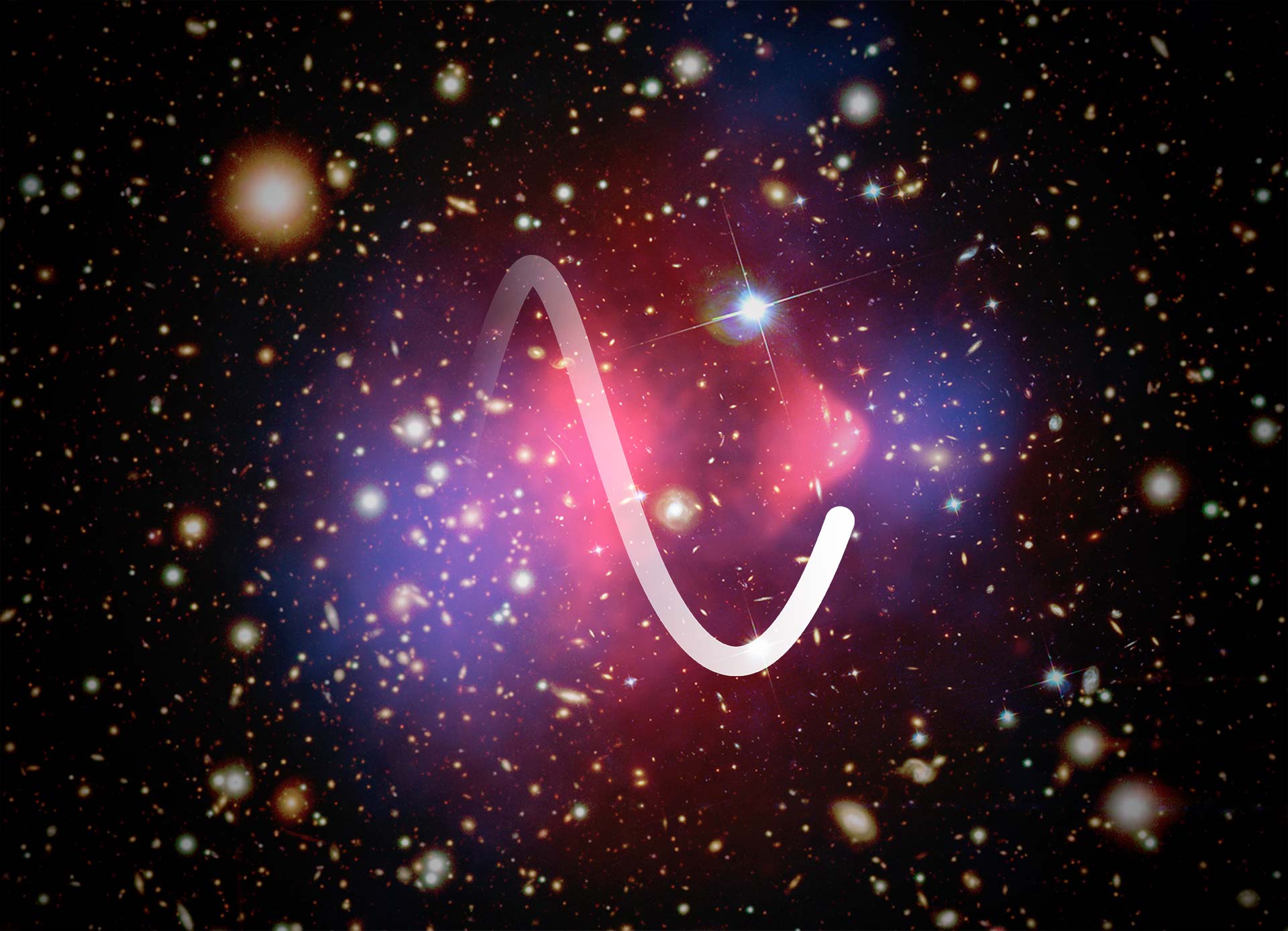
Pushing the intensity and energy frontiers
Particle physics has arrived at an important moment in its history. The discovery of a light Higgs boson - with a mass of 125 GeV - opens a new era, clearing the decks for a new phase of exploration of physics beyond the Standard Model. Particle physics must continue its investigations, in the broadest possible way, with a mix of radical improvements in sensitivity, precision, and energy range.
A future circular electron-positron collider (FCC-ee) implemented in stages, from 90 to 360 GeV, allowing to study in depth the interactions of the four heaviest particles known today (i.e. the Z and W bosons, the Higgs boson and the top quark).
FCC-ee offers ideal conditions (luminosity, energy calibration, a.o) for the study of these particles with a flurry of opportunities for precision measurements, searches for rare or forbidden processes, and the possible discovery of feebly coupled particles. FCC-ee is also the perfect springboard for a 100 TeV proton collider (FCC-hh), for which it provides a great part of the infrastructure.
The complementary and synergistic physics programs of these two machines offer a uniquely powerful long-term vision. This vision is the backbone of the 2020 European Strategy for Particle Physics.
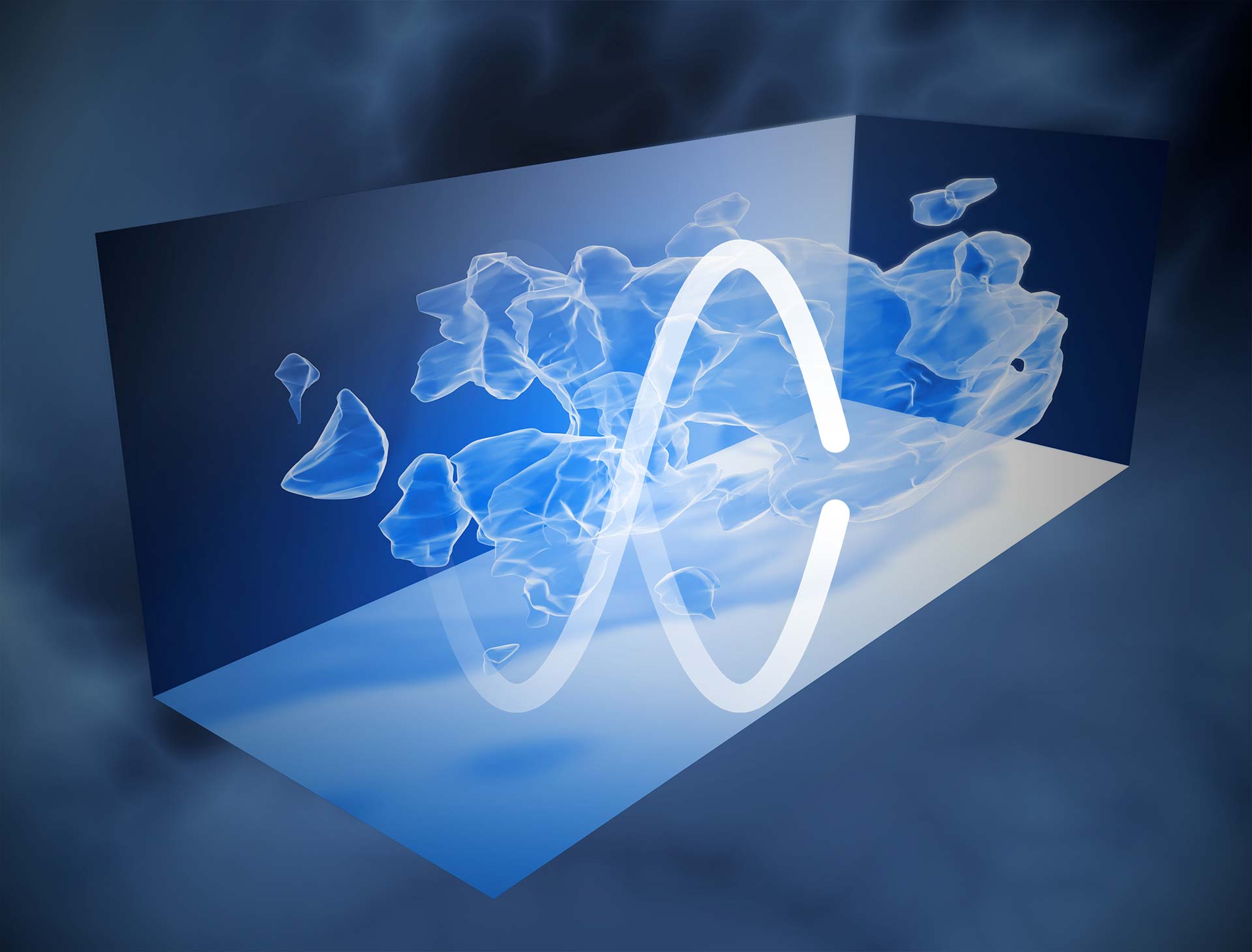
Searching for dark matter?
Several astronomical observations indicate that the Universe contains more matter than seen by the naked eye. Visible matter only accounts for 5% of the content of the universe! Another 27%, is made up of dark matter, which, with its gravitational pull, holds galaxies and galaxy clusters together.
Ordinary matter, known as baryonic matter, is composed of protons, neutrons and electrons and is described by the Standard Model. Dark matter candidates arise frequently in theories that suggest physics beyond the Standard Model while it could be made of new, yet undiscovered particles.
No experiment, at colliders or otherwise, can probe the full range of dark matter (DM) masses allowed by astrophysical observations. However there is a very broad class of models for which theory motivates dark matter candidates with masses in the range of GeV to few tens of TeV. The FCC would break new ground in the search for dark matter in the form of weakly interacting massive particles, by covering a wide array of potential signals predicted by either production of dark matter, or production of the particles mediating its interactions with ordinary matter. FCC-ee and FCC-hh offer complementary ways to search for dark matter that could consist of lighter particles (i.e. sterile neutrinos) or could be produced in the decays of the Higgs boson.
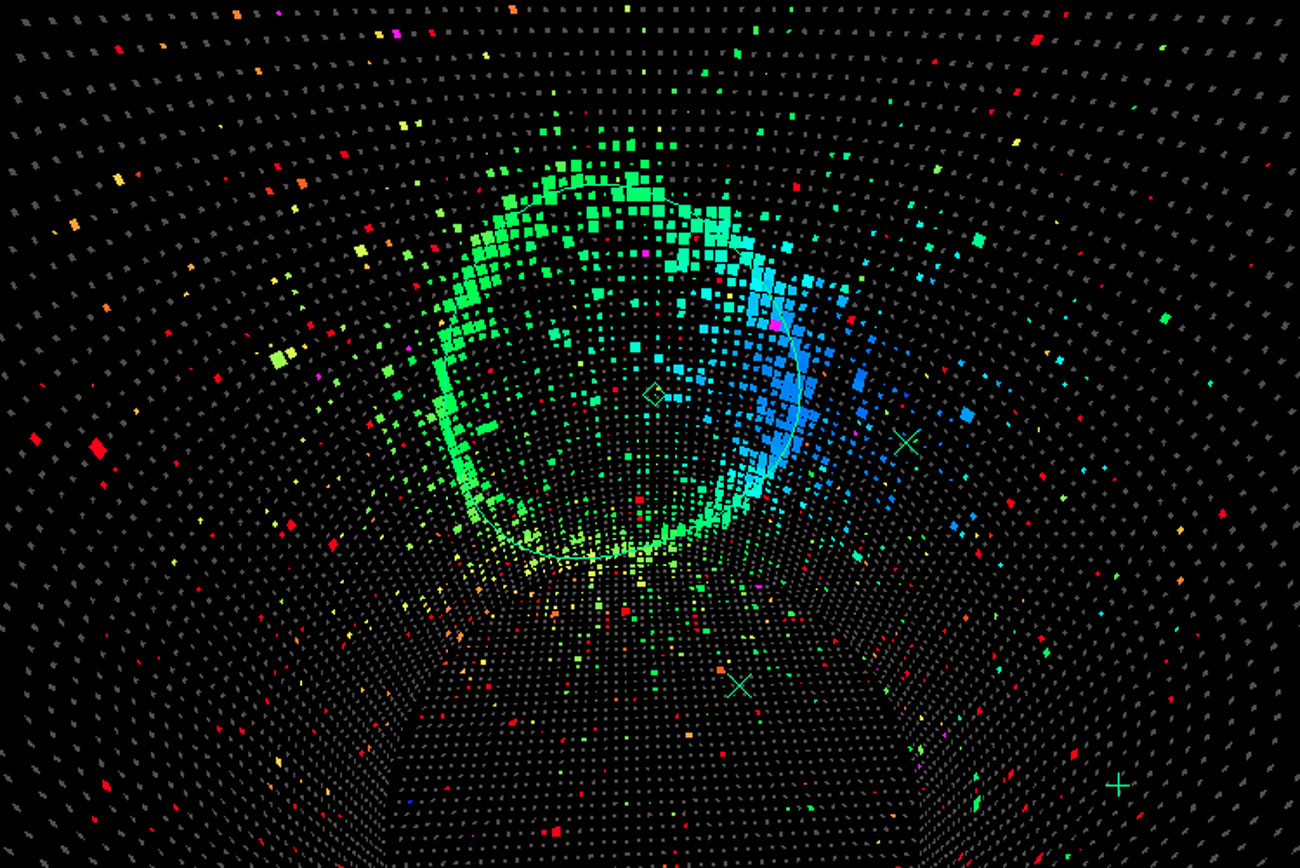
Why do neutrinos have masses?
One of the fundamental experimental questions in physics today is how neutrinos acquire their mass as this is not predicted by the Standard Model.
In the first decade of the new millennium the modern neutrino experiments provided the first evidence for the phenomena called neutrino oscillations, implying that the neutrinos have a small mass. According to the Standard Model, neutrinos are massless particles, thus this discovery marked the first crack of the Standard Model. In 2015, Takaaki Kajita and Arthur B. McDonald were awarded the Nobel Prize in Physics for “their key contributions to the experiments which demonstrated that neutrinos change identities”.
The crack isn’t big enough to completely shatter the Standard Model as we do not know what generates the tiny neutrino masses. FCCee has the unique opportunity to shed light on this topic. Neutrino masses can be generated by their heavy counter partners called Heavy Neutral Leptons which, if they exist, will be produced via the decay of Z bosons. During its first stage of operation, FCCee will produce trillions of Z bosons thus allowing it to search for these particles up to the electroweak scale.
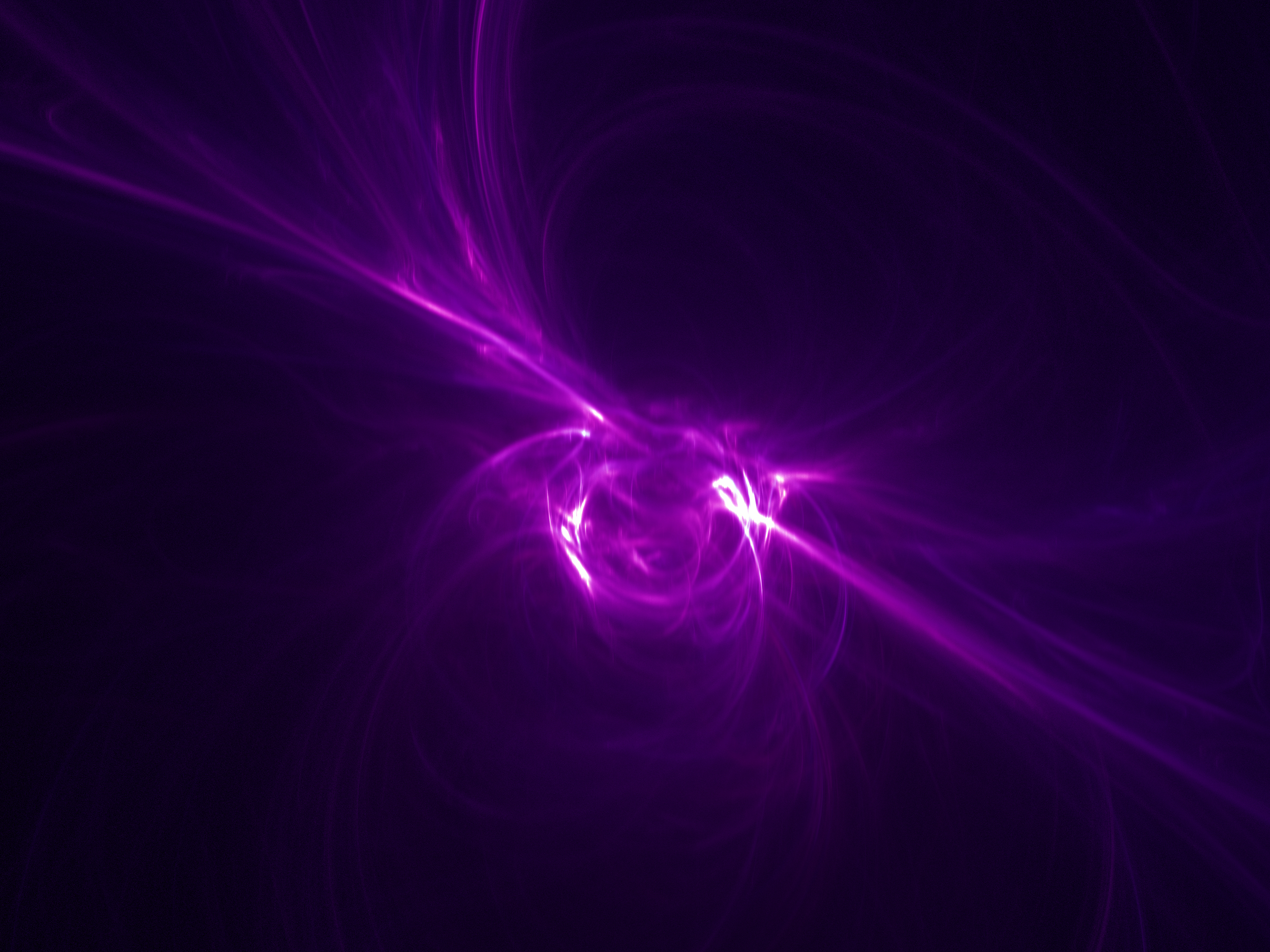
Why there is more matter than antimatter in the Universe?
Another piece of experimental evidence pointing to the need to continue exploring the physics of subatomic particles is the prevalence of matter over antimatter. Almost 14 billion years ago the Big Bang - the event that gave birth to the universe that we know - created equal amounts of matter and antimatter. Yet currently the universe is made entirely from matter, and its counterpart is nowhere to be seen. The question: where did the antimatter disappear to, is one of the most important ones in particle physics that has not been answered so far. Explaining the origin of the cosmic matter-antimatter asymmetry is a challenge at the forefront of particle physics.
We know that nature can create matter-antimatter imbalance via the so-called CP violation. The problem is that the amount of CP violation that we measure in current experiments is insufficient to explain the state of our universe. Therefore the common idea addressing this imbalance is to assume the existence of a new source of CP-asymmetry. FCCee will be capable of probing new sources of CP-violations thanks to its enormous integrated luminosity. Combined with the FCC-hh, the FCC should conclusively probe new states required by a strong first order phase transition that favoured matter to prevail in the Universe.
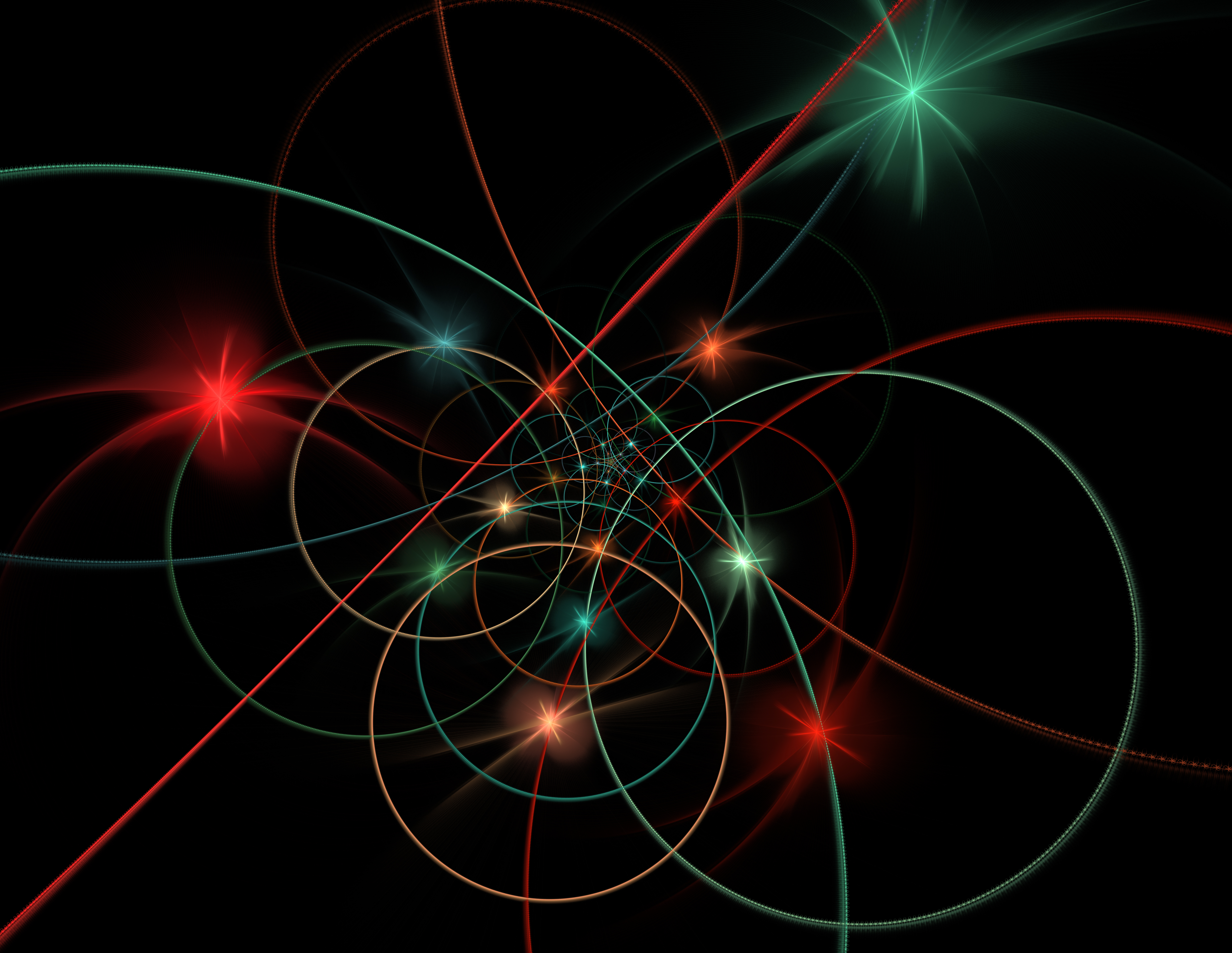
Looking for Supersymmetry?
Experiments have confirmed the Standard Model of particle physics time and again. But the model is incomplete. Among other features, it cannot explain dark matter, or the small mass of the Higgs boson or why the forces acting between particles do not unify at high energies. These are some of the questions that supersymmetry could address. The simplest supersymmetric theories—those that best explain the Higgs boson—predict a wealth of supersymmetric particles that should be within the reach of the LHC.
Theorists introduced supersymmetry in the 1960s to connect the two basic types of particles seen in nature, called fermions and bosons. Roughly speaking, fermions are the constituents of matter, whereas bosons are the carriers of the fundamental mental forces. Supersymmetry would give every known boson a heavy “superpartner” that is a fermion and every known fermion a heavy partner that is a boson
The lack of signs, thus far, of supersymmetric particles at the LHC implies SUSY, as interesting as it may be, does not appear to describe our Universe. Future searches at lepton and proton colliders would further constrain any viable scenarios and put progressively tighter bounds to SUSY candidate particles. Searches could profit from data collected at the FCCs as they will allow better discrimination of the Standard Model backgrounds but also deliver more information for event reconstruction. Data collected during the FCC’s experimental programme would help test the different manifestations of this theory and guide theoretical thinking